Discussion regarding the art and science of creating holes of low entropy, shifting them around,
and then filling them back up to operate some widget.
28 November 2005
Energy Efficiency leads to ... Wealth?
This is not so much a conscious decision people make to consume more energy but rather a move towards enriching ones lifestyle. Wealth is not the number of digits of fiat currency one has in their bank account and stock portfolio. That would be an overly simplistic viewpoint. The wealth of an individual is argely be determined by the services they have access to and can afford. By most measures I am wealthier than the Rockefellers of the late 19th century. I have access to faster and far safer transport, superior health care, and the marvel of modern internet communication, yet I am a graduate student and live below my country's version of the poverty line. All of my wealth is due to the efforts of scientists and engineers before me who have developed the technology and infrastructure that I now enjoy.
This issue becomes important when we try to evaluate the impact of improved efficiency on the production of greenhouse cases or oil consumption. Would a significant effort to increase the fuel efficiency of the personal vehicle fleet lead to a real reduction in oil consumption? Or would the reduction in demand drive a price drop in the cost of various forms of energy and in turn generate more wealth?
At what price point does efficiency begin to effect conservation positively?
I am not an economist (and economists have a terrible track record on predicting the future) but I suspect the answer lies somewhere between demand elasticity and fossil fuel depletion rates. I would suggest that the more inelastic the demand for oil the greater the long term reduction in consumption with increased prices. A high degree of inelasticity suggests that the system has a great deal of inertia and that much of the consumption is not so much voluntary but necessary to produce our wealth. In comparison shifts in elastic economy would be more temporary. Fossil fuel depletion is important because it dictates supply or will do so very shortly.
While improvements in efficiency certainly seem to present the lowest hanging fruit on the quest for sustainability they will likely only have a transient impact on energy consumption. Long term solutions to our energy situation will require a change in supply rather than demand.
23 November 2005
Sam Passes On
sniff
In other news I have been catching up on my sleep since finishing my written thesis and all the bureaucracy associated with actually organizing my oral defense. Also Ben at theWatt.com has gone AWOL at the National Research Council labs so I have been trying to keep that site from becoming moribund. I promise to finish off some of the half-complete posts sitting in my box sometime soon. Of course, if my definition of soon is not the same as yours, too bad.
13 November 2005
Embedded Energy Metric
This energy investment in a product is known as the embedded energy. It is equivalent of capital investment in the money dimension. For example, in order to compare wind turbines to a combined-cycle thermal-electricity plant we should compare not only the CO2 offset by using wind rather than natural gas fuel to produce electricity but also the energy invested in the construction of the infrastructure as well. The steel and concrete in a wind turbine has an associated energy and CO2 production embedded into it during its manufacture. This embedded energy must be amortized over the lifespan of the turbine in order to properly determine the CO2 emissions that are offset.
The trick is how to determine the embedded energy in a product with adequate accuracy? To a certain extent this issue has stalled my investigation into the use of anerobic bioreactors to produce biogas from crop waste along with difficulties finding data on reaction rates as a function of temperature. Well, this being the blogosphere, an order of magnitude calculation on the back of an envelope should suffice. My thought is that if I could establish a metric for some common materials then I could simply estimate the embedded energy based on the mass of material in a product. The embedded energy problem is potentially simplified but it still remains challenging. Just try to find the mass of an air conditioner on a vendor's webpage, for example.
As a rough measure every material in the energy world can be subdivided into the following categories:
- Steel
- Aluminium
- Copper
- Plastic (polyethylene)
- Silicon
- Concrete
Rather than try to determine the energy embedded in a particular alloy I am hoping that StatsCan will have industry-wide figures for both production and energy consumption which will result in a more inclusive result. Hopefully I will be able to arrive at a reasonable set of metrics by the end of the week. Of course it would be desirable to actually check the results. Most likely there will be a need to introduce a fudge factor to account for the transformation of sheet steel into an automobile.
12 November 2005
Simple Solutions for Truck Freight Efficiency
http://www.greencarcongress.com/2005/11/new_boat_tail_d.html
This just goes show how simple it could be to improve the efficiency of freight transport by truck. There's a number of other improvements that could be refited to existing truck fleets, like shrouding the tires and creating a flexible connector between the cab and trailer. Once it became clear that truck manufacturers weren't about to make improvements to the aerodynamics of their vehicles the government should have stepped in with legislation. Sometimes industries need a carrot to get them over a hill. In this case they need a kick in the ass to get them moving in the right direction.
01 November 2005
Solar Thermal Cooling
US peak electricity consumption occurs during the summer months when the demand for cooling is high and the efficiency of heat pumps drops with the higher ambient temperatures. Air conditioning constitutes approximately 15 % of annual US electricity consumption. However, the proportion of demand on peak days in hot states is in fact much higher.
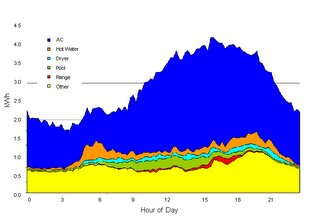
Supplying peak power is very expensive for utilities because they must build the infrastructure to cover peak demand which otherwise sits around underutilized. As a potential means of peak shaving we can examine the use of solar power to provide cooling. In this case utilities could be persuaded to offer businesses and home owners incentives to install such systems because it would improve their bottom line. The introduction of net metering with variable electricity prices could also provide an impetus. In the current environment of fixed rate electricity the consumer has little reason to purchase such a system.
There are two practical approaches:
- Pair a photovoltaic array with a standard air conditioning unit.
- Pair a solar thermal collector with an aborption chiller.
A solar thermal system would use a collector to provide hot water for a single effect absorption chiller. Absorption chillers differ from common compression chillers in that cooling is driven by heat energy rather than mechanical energy. Aside from the plumping, several pieces of ancillary equipment are necessary including a controller, a pump, and a drain-back/expansion tank. Both systems are capable of providing hot water in addition to their cooling functions. For the PV system, a Heat Recovery Unit (HRU) would be used to pre-warm the water using waste heat from the heat pump.
Some further explanation of absorption chillers is warranted given that they are relatively uncommon. An absorption chiller transfers thermal energy from the heat source to the heat sink through an absorbent fluid and a refrigerant. The absorption chiller accomplishes its refrigerative effect by absorbing and then releasing water vapor into and out of a lithium bromide or ammonia/water solution. The process begins as heat is applied at the generator and water vapor is driven off to a condenser. The cooled water vapor then passes through an expansion valve where the pressure reduces. The low-pressure water vapor then enters an evaporator, where ambient heat is added from a load and the actual cooling takes place. The heated, low-pressure vapor returns to the absorber, where it recombines with the lithium bromide and becomes a low-pressure liquid. This low-pressure solution is pumped to a higher pressure and into the generator to repeat the process.
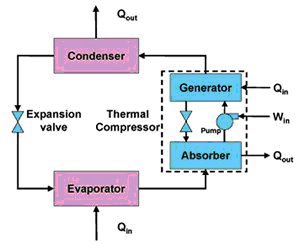
The performance of air conditioning systems is expressed by their coefficient of performance (COP). COP determines how many units of cooling/heating you get for every unit of energy you put in. Because units move heat the COP can be in excess of one. A typical value for a air conditioning heat pump is about 3.5 compared to only 0.7 for a absorption chiller.
The COP of an absorption chiller is heavily dependant on the applied temperature. If the solar thermal system is only capable of producing tepid water it will not function effectively. For my analysis I will assume that the absorption chiller requires an input temperature of 85 oC compared to the typically 55 - 60 oC required for residential hot water. The obvious conclusion from this is that the cheap unglazed flat-plate solar thermal systems used to warm pool water aren't going to provide the temperatures we need. Instead, we have to move up to evacuated tube collectors.
Evacuated tube solar thermal collectors are sold by companies such as www.apricus-solar.com. They incorporate a number of improvements to increase their efficiency and maximum operating temperature:
- The absorber is insulated from the air by a vacuum. This greatly decreases convective losses to the wind.
- The absorber is coating with a spectrally selective surface. Such a surface has a high absorption coefficient (α ~ 0.9 – 0.95) in the visible wavelengths (550 nm) but a low emissivity coefficient (ε ~ 0.02 – 0.08) in the far infra-red (10,000 nm). This reduces radiation losses to a minimum.
- Heat is transferred from the absorber to the working fluid by means of a heat pipe. A heat pipe is filled with a partial pressure of water, such that it boils at 30 °C. When the water evaporates, it convects up to a heat exchanger where it condenses, transferring its energy to the working fluid. The effective thermal conductance is roughly 10,000 times that of a solid copper bar. The heat pipe also has a diode function, in that if it is not hot enough to evaporate the water, no convection will occur.
PV/Heat Pump: 0.125 * 3.5 = 0.44
Evacuated Solar-thermal/Absorption Chiller: 0.65 * 0.7 = 0.45
Amusingly they come out to being almost exactly the same. Assumably the inverter losses and parasitic heat losses are small and roughly equivalent. Presumably if you did an entropy analysis this would fall out quite naturally. Since the performance of each system is equivalent it is going to come down to cost.
If I size a 5000 W cooling system (for peak production at 1000 W/m2 insolation) for a home this is what I get:
Photovoltaic | Solar Thermal | ||
Component | Normalized Cost (US$/Wcool) | Component | Normalized Cost (US$/Wcool) |
PV Array | 1.21 | Solar Collector | 1.99 |
Heat Pump | 0.16 | Absorption Chiller | 0.32 |
Inverter | 0.34 | Pump | 0.037 |
| Controller | 0.079 | |
Drain-Back Tank | 0.064 | ||
Total | 1.71 | Total | 2.49 |
This is ignoring mounts, plumbing and wiring for both the solar thermal collector and PV array. As we can see it is the cost of the evacuated solar collector pushing it above the PV solution. The expensive nature of evacuated solar thermal tubes is probably due to both their complexity and their low production volumes. Since they don't appear to be superior in this role now it seems unlikely that they would ever be able to catch up.
PV / Heat Pump System
Photovoltaic Panel
Name: Sharp ND-167U1
Peak Power: 167 W at 1000 W/m2 insolation
Module Efficiency 12.6 %
Area: 1.33 m
List Price: US$ 688.97
Heat Pump Chiller
Type: LG model LWK0710WGL
Cooling Power: 2051 W (7000 btu)
Coefficient of Performance: 3.43
Electrical Consumption: 600 W
List Price: US$ 330
Inverter
Type: Grid inter-tie
Name: Solectria PVI 1800W
Continuous Power Input Rating: 1980 W
Recommended Max PV Array Power: 2200 W
Continuous Power Output Rating: 1800 W
List Price: US$ 1731.25
Solar Thermal / Absorption Chiller System
Solar Collector
Name: Apricus 30 Tube Collector
Gathering Area: 2.4 m2
Estimated Peak Power Generation: 1536 W
Absorption Chiller
Name: Yakaza WFC-10
Cooling Power: 10 tons
Coefficient of Performance: 0.7
List Price: unknown (generally 2x that of heat pump)
Controller
Name: Apricus Sentinel-Pro controller
List Price: US $395
Pump
Name: Grundfos Stainless UP15-18SU
Power: 1/25 hp
List Price: $184
Drain-back/Expansion Tank
Name: Alternate Energy Technologies DB-10
Capacity: 10 gallons
List Price: $322
27 October 2005
Woohoo!
I am currently enjoying copious quantities of Hoegaarden beer so don't expect any intelligent commentary for tonight.
22 October 2005
A Quick Swipe at "Free" Hydrogen
A company called Alternate Energy Corporation claims to have some process that reacts water with metals to produce hydrogen gas. A quick browse of their website should set off alarm bells for anyone -- the distinct lack of any details on their system for example. Over at theWatt.com we've found some references to systems that burn aluminium to produce hydrogen. Aluminium won't react with water; you must introduce some acid or base to make the process run. Still, let's just imagine that we have the following irreversible reaction with zero enthalpy.
2 Al + 3H2O → Al2O3 + 3 H2
Given that you input 2 moles of Al for 6 moles of H it would require 9 kg of Aluminium to produce 1 kg of hydrogen. But the manufacture of aluminium is extremely energy intensive!!! Running the Aluminum Association's numbers I come up with 147.7 MJ/kg. This means you need 1329.3 MJ to produce one kilogram of hydrogen with an energy content of 142 MJ. This gives me an ERR of about 10 %. Whee.
If I ran a similarly fictitious reaction with iron I would need 18.6 kg of iron to make a kilogram of hydrogen. You can guess where this is going.
Now if we just burned the aluminium with oxygen in air... (we would have rocket fuel)
20 October 2005
Hydrogen Debunked
http://www.efcf.com/reports/E15.pdf
http://www.efcf.com/reports/E16.ppt
I made a PDF version of E16 for those of you without the latest version of Powerpoint.
19 October 2005
A Candle that Burns Half as Bright Burns Twice as Long
The trucking industry is one that has remained practically devoid of technological advances over the past thirty plus years. The fuel economy of transport trucks hasn't improved at all since 1980 (as far back as I have data). This is pretty surprising given that the cost of fuel is currently about twice the pay of the driver when driving at highway speeds. The mean pay for a trucker is $16.00/hr whereas a 5 mpg truck driving at 55 mph burns around $33.00 of diesel. I don't know what exactly to make of the lack of imagination.
I'll use the following assumptions for my hypothetical truck:
Loaded Mass: 35 000 kg
Frontal Area: 12 m2
Length: 15 m
Rolling Resistance Coefficient: 0.007
Rolling Resistance: 2400 N
Drag Coefficient: 0.7
Drag @ 110 km/h: 4500 N
Drag @ 80 km/h: 2400 N
The first and most obvious point of improvement is the aerodynamics. The drag coefficient for semi-tractor/trailers runs about 0.7 which is nowhere near the level obtained by modern automobiles (0.3). There's no real reason for trucks to be worse. In fact the Bladerunner concept shows a great deal of improvement in aerodynamics. They lowered the profile, improved the nose, eliminated the gap between the tractor and trailer, added aerodynamics to the wheels, and smoothed off all right angles.
Drag Coefficient: 0.3
Drag @ 110 km/h: 2000 N
Drag @ 80 km/h: 1000 N
This yields a pretty impressive drop from 6900 N overall resistance at 110 km/h to 4400 N which should result in a 55 % improvement in fuel economy. This can be achieved by cleaning up the aerodynamics of trucks to passenger car levels. I'm not taking into account the lower profile of the Bladerunner which would reduce the frontal area exposed to drag.
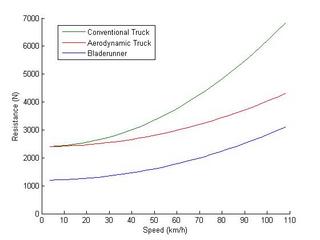
Now to examine the viability of running trucks on rails. The Bladerunner concept has a number of advantages by using the pneumatic tires as the power wheels and the steel bogies for load bearing. It breaks much faster and allows for much higher traffic density on the rail line. However this does come at a price. The rubber tires will no doubt impose their own rolling resistance. The Bladerunner article claims it halves the rolling resistance in exchange for 500 kg of extra mass for the rail bogies. This seems reasonable given that trains are about 3 - 6 times more efficient on the rolling resistance end. This would drop the rolling resistance from 2400 N to 1220 N. Practically speaking the extra mass of the bogies is irrelevant.
So if we compare our aerodynamic conventional truck to our aerodynamic Bladerunner the overall resistance is 4400 N and 3200 N respectively. The improvement in fuel economy is now 37.5 % which may be respectable enough to be economical. The full Bladerunner (3200 N) versus a contemporary (1970-tech) truck (6900 N) represents a fairly vast 115 % improvement. Cutting fuel consumption from truck shipping in half would represent a great reduction in the demand for petroleum.
Practically speaking in order to get the absolute best performance you would want Bladerunners to run in convoys so close together that they would be able to draft off each other. In this case the leading truck would have the hardest drive and the others would cut a break off his slipstream. Since this isn't exactly safe nor fair for the leading vehicle the obvious solution is to actually couple the vehicles in a convoy into a road/rail train. This would require a good communication system amongst the drivers if you wanted to be able to shift trucks in and out of a convoy on the move but it could certainly be worth it. If the vehicles were also hybrids driven by electrical motors then it would also be possible for the trailing vehicles to transfer power to the leading vehicle. In this case the following vehicles could completely retract their rubber wheels from the ground and travel solely on their steel bogies.
The other question to examine is how much is a driver's time worth? We can clearly see from the numbers that from a fuel economy perspective driving 30 km/h slower cuts the drag approximately in half. The disadvantage is that the driver must be paid more to drive more hours and the load will take a little longer to arrive. We can probably discount the amortization of the vehicle itself because that will be based on distance and not time traveled.
At a pay rate of $16.00/hr the marginal cost of slowing down 30 km/h is $6/hr or $0.075/km. With our current bad performance truck fleet slowing down by 30 km/h will shave off about 2100 N resistance, or 2.1 MJ/km. If we have a 30 % tank-to-wheel efficient engine (high side estimate) then we are reducing our burning of diesel by 7.0MJ/km. Diesel has an energy content of 46 MJ/kg and costs about $0.70/kg in the States. Hence our savings for traveling slower works out to $0.107/km. We're saving almost three cents a kilometer by driving slower. These numbers don't work out quite so well for the Bladerunner obviously. The efficiency gains will offset the economics to allow driving at a higher speed.
UPDATE: A quick check of my resistance numbers (6900 N at 30 % engine efficiency) show a burn rate of 23 MJ/km or half a kilogram of fuel. This works out to 4.3 mpg which is less than the fleet average of 5.3 mpg. This would be the case for driving in a straight line without any traffic or stops or hills etc. On the other hand, my truck is heavily loaded. The numbers seem reasonable to me although they could no doubt use some improvement.
Stuart Staniford at the Oil Drum put up a post about the fleet fuel economy numbers. I encourage readers in particular to look at the graph showing fuel economy for trucks. It's flat.
17 October 2005
Basic Bateria Flatulence
There are two basic pathways. The first is the production of hydrogen directly from polymers. The second is a longer process that breaks down polymers to acetate. Methanogenesis can consume either CO2 and H2 or acetate ion (CH3CO2) which also produces CO2 as a byproduct. In addition to hydrogen and acetate some other fermentation products such as methanol can also be transformed into methane.
The acetate pathway is the longer of the two. The first step is the basic fermentation of proteins, fats, and carbohydrates into smaller chunks. The next step is to break down the intermediate products into acetate(CH3COO-) and acetic acid (CH3COOH). The pH of the solution can become unbalanced at this point which is disadvantageous to the consumption of acetane into methane.
The basic reaction is:
C2H4O2 → CH4 + CO2
The hydrogen pathway can consume most forms of biological material. It basic disadvantage is that the enzyme used rapidly reduces in effectiveness in the presence of hydrogen. The very production of hydrogen acts to stall the process. As such the hydrogen must be quickly removed from the system. Diffusion is generally slow but feeding the hydrogen into another chemical reaction can consume it quickly. Because the product is hydrogen gas there has been considerable research into this pathway. Research has focused on genetically modifying the enzyme to operate in the presence of hydrogen or engineering improvements to increase the yield. Methanogens (methane production bacteria) can react H2 with CO2 to form methane. This will produce oxygen gas which is not desirable in the anaerobic environment.
The basic reaction is:
CO2 + 2H2 → CH4 + O2
Methanogensis can only take place in an anaerobic environment. Maintaining an anaerobic environment not only protects the oxygen adverse bacteria but also prevents the growth of oxygen breathing methane burners.
The rate of methane production is highly dependant on pH and temperature. Most methanogens (mesophiles) prefer a temperature between 30 - 40 o and a pH near neutral (7.0) or slightly alkaline. My inability to find temperature and pH dependence is one of my stumbling points at the moment.
Methane producers are not the only bacteria in the pond that consume hydrogen and acetate. In particular, sulfur and nitrogen fixing bacteria both out compete methanogens for reactants. Sulfur fixing bacteria like to produce Hydrogen Sulfide gas (H2S) which then bubbles off and should be separated from the biogas flow. On the other hand, nitrogen fixing bacteria tend to produce ammonium ion (NH4+) which then finds something else to bond to. Ideally one might want to search for a bacterium that fixes sulfur into a solid form that is suitable for soil fertilization. Principally one could find data on the N and S content for Canola meal and figure the number of moles of H2 consumed.
Just as an aside, I did find some research on the production of butanol from biomass instead of ethanol. I don't have on-line access to this research so I can't easily review it but it is interesting nonetheless.
16 October 2005
On The Rails
I thought it would be valuable to look at the major reasons why trains are more efficient than trucks. There are three main areas to look at:
- Air drag
- Rolling resistance
- Transient accelerations
Fd = 1/2 cdρAcv2
where Fd is the drag in Newtons, cd is the coefficient of drag, ρ is the density of air (1.17 kg/m3), Ac is the area cross-section, and v is the velocity in meters per second. For cargo transportation we should normalize the coefficient of drag by the ratio of the cross-sectional area to the overall length of the cargo carrier.
The rolling resistance on the other hand is not very dependant on speed. Drag is usually expressed as
Fr = crgm
where Fr is the rolling resistance in Newtons, cr is the coefficient of rolling resistance, g is the acceleration of gravity (9.81 m/s2) and m is the mass in kilograms.
Transient accelerations basically refers to traffic and hills. Trains have right of way so they don't have to stop and yield to other traffic at any time. Their rail lines also tend to go through or around hills rather than over them. As such they do not waste energy by speeding up or slowing down unnecessarily. Regenerative braking can mitigate this issue to a large extent.
Let's take a look at an example for a car, semi-truck, and a 50-car train.
Transport | Car | Semi | Train |
cd | 0.3 | 0.7 | 2 |
cr | 0.01 | 0.007 | 0.0015 |
L:A ratio | 0.5/m | 3.5/m | 175/m |
Trucks are less aerodynamically efficient than cars for a variety of reasons: their blunt front-end, many exposed turning wheels, gap between the cab and trailer, and the sharp back end. I don't really know how aerodynamically efficient a train is but I can guess that it basically creates its own wind. On rolling resistance trucks have a minor advantage over cars because they operate at higher pneumatic pressure. The steel wheels on trains don't deform at all so they are considerably superior.
The key point is in fact the length to cross section area ratio and its impact on aerodynamic drag. If we normalize the coefficient of drag by the L:A ratio the results for cars and trucks are on the same order of magnitude, 0.6 and 0.2 respectively. The train normalized drag coefficient is dramatically lower at 0.01. Essentially because the front cars will push the wind the remaining cars get a free ride against air resistance. This reduces the overall air resistance on a train per unit mass of cargo regardless of its aerodynamic shape.
If we assume a mass of 35,000 kg for the semi and 2,500,000 kg for the train we can graph an estimate for the rolling and drag resistance:


The resistance at 108 km/h (30 m/s) works out to 0.16 N/kg for a truck and 0.02 N/kg for a train. In this estimate the train is about eight times more efficient. That matches well with generally quoted values. What's more significant is how the rolling resistance forms a much greater proportion of losses for the train compared to the truck. This underscores how the long length of the train gives it an advantage on the drag side of the equation.
11 October 2005
Photoelectrochemical Alternatives
The Energy Blog had a post detailing electrochemical photovoltaic cells that are capable of directly producing Hydrogen gas. It has a pair of advantages:
- It reduces the electron current on the PV cell by consuming the electrons. This should result in a decrease of the recombination of electron-hole pairs.
- The electrolyzer is effectively integrated into the system.
I do not think too highly of this concept. It makes several requirements of photovoltaic systems:
- The active semiconductor material must be capable of resisting corrosion from the hydrogen.
- The semiconductor's band gap must be well matched to the radiation spectrum of the sun.
- The redox potential of water must be well matched to the band edges of the semiconductor.
Simply put, there are no known materials that can meet all three criteria simultaneously. The solution seems to be to produce triple-junction cells to produce mediocre hydrogen conversion efficiencies. I remain unimpressed. Nature simply has not cooperated in providing an appropriate material.
If we take off our hydrogen goggles for a second an alternate solution presents itself. Instead of searching for a suitable semiconductor material perhaps we should search for an appropriate reversible reduction-oxidation reaction. I am thinking specifically of flow battery concepts.
The big name in flow batteries is VRB Power of Vancouver. They build a type of flow battery that uses Vanadium electrolyte to store power. In addition to the Vanadium concept, there are many other redox reactions that have been implemented such as Zinc-Bromine and Sodium Polysulfide Bromine. Such a coupled electrolytic photochemical system would probably still require a membrane to separate the positive and negatively charged electrolytes. Unlike the water reduction, the products won't bubble off as a gas; bromine systems may be an exception.
By introducing another degree of freedom by allowing the redox reaction the number of possible permutations between semiconductor and redox will greatly increase. This increases the likelihood that a natural match will be found to create a solar energy solution coupled to a storage technology.
There's no need for storage coupled to solar energy for on-grid applications yet. On the other hand, off-grid applications do tend to have very large battery packs. The main question is would the extra cost of the flow battery be offset by the superior energy return rate? My gut feeling is no -- off-grid applications are typically very small with electronics designed to minimize power drain.
10 October 2005
Basic Biodiesel Economics
A list of Canola prices can be found here, denominated in Canadian dollars. To summarize since 2000:
Year | Crop Average Canola Oil Price (C$/ton) | Crop Average Canola Meal Price (C$/ton) |
2000 | 568.84 | 156.27 |
2001 | 485.54 | 205.05 |
2002 | 633.10 | 222.80 |
2003 | 813.70 | 214.38 |
2004 | 811.16 | 240.76 |
2005 | 660.22 | 205.40 |
Mean | 662.09 | 207.44 |
Standard Deviation | 131.09 | 28.36 |
For the degummed oil, one metric ton is approximately 1000 L of diesel equivalent or 220 US gallons. Given an exchange rate of about C$0.85/US$, we can find that the basic input price for the oil should be around $C0.66/L or US$2.5/gallon. The cost to process vegetable oil into esters needs to be added to that to determine a final price for biodiesel. Without tax breaks for biodiesel it is not competitive with the fossil fuels. The price of crude will have to approximately double for biodiesel to be competitive.
The story for the meal to methane is slightly different. If we get 500 kg of methane gas from every 1000 kg of meal input, we'll get out about 28 GJ worth of gas. That works out to about US$6/mcf for natural gas which is about half the going rate in North America. If the bioreactor could be built sufficiently cheap this could end up being cost effective now. This is ignoring the inherent value in the remaining sludge as fertilizer.
How about Kyoto carbon credits you ask? Methane will produce about 2.75 tons of CO2 per ton burned. At US$35/ton(CO2) that's about a $50 carbon credit per ton of meal. Alternatively it's US$1.70/mcf. (Not sure why I'm pricing carbon credits in US dollars here...)
For methyl oleate ester you have 38 hydrogens, 19 carbons, and 2 oxygens per ~300 amu molecule. The fuel to CO2 multiplier is about the same as methane at 2.8. On a mass basis biodiesel as 87 % the energy content of fossil diesel. We can apply for a Kyoto credit of about US$85 per ton of Canola we transesterify into biodiesel. This works out to approximately US$0.40/gallon. So the Kyoto credits can make a substantial impact on the cost of biodiesel for those countries that have signed the treaty and are serious about implementing it. It might be useful here to also take a look at costs for other smog and acid rain pollutants which the USA does trade in.
These are some very rough calculations but they show about where the break even points are for biofuels. What's most interesting to me is that because natural (fossil) gas is so expensive biogas may be the most economically viable biofuel in North America at the moment. Surely there are cheaper sources of biomass available than high-protein canola meal. Since the gas situation isn't likely to improve soon investments in this area are plausible. Biodiesel, on the other hand, isn't ready for the big time quite yet.
09 October 2005
Can You Smell the Freedom ExhaustTM?
Generally speaking in we want the oil with the lowest surface tension so that it will flow through the fuel injection system easily and atomize in the combustion chamber well. This means we want to use unsaturated, short chain fatty acid. If we compared oleic acid with its saturated version, stearic acid, we can the difference in viscosity and melting temperature. Also we could compare oleic acid, with 18 carbon atoms, to erucic acid with 22 carbon atoms. Actual distributions of fatty acids in crop types can be seen in this table from Iowa State University. The table shows that Soya has a 8-15 % saturate content, versus 5.6 % for Canola or 11.2 % for traditional rapeseed.
Canola is a cultivar of rapeseed developed in Canada in the 1970s. It specifically bred out eruric acid (long-chain) content and increased the content of 18-chain unsaturated oils. This turns out to be to an advantage for the production of clean burning biodiesel. Canola also bred out glucosinolate content which may lower the pest resistance of the crop. On the other hand, the glucosinolate molecule contains sulfur and nitrogen. Canola has been genetically engineering by firms like Monsato to be herbicide resistant in an effort to increase crop yields. Unfortunately canola cross-pollinates between fields so issues with so called monster food aren't avoided in biodiesel production.
The check variety of Canola (to which other types are compared) is 46A65. It has an average yield of 49 bushels/acre which using this conversion table works out to 2.746 tonnes of biomass per hectare in middle and short growing zones. The longer growing system zones (mostly in Manitoba) can show yields up to 28 % higher. The highest yielding variety from Canola Council trials appears to be a set of Bayer CropScience varieties (5020, 5030, 5070) all running approximately 125 % yields. This is a herbicide resistant crop running the Liberty system. The yield would then be 3.432 tonnes/hectare.
The next step is to examine the composition of Canola. Government statistics show that the oil content is approximately 43 %. There are a variety of ways of extracting the oil from the plant which I will probably discuss at a later date. The remaining biomass (canola seedcake) has a number of potential applications. It can be composted and reapplied to the field but this does not take advantage of the energy stored in the biomass material. It can be sold as high-protein animal feed. It can be burned as biomass to produce heat and electricity as an alternative to coal. It can be fermented into alcohol fuels but the energy return on fermentation is poor. My alternative is to compost the biomass anerobically in water to gather methane from the biomass while retaining the nutrient content that would go up in smoke if the material was burned.
The overall composition of the canola harvest can be estimated by looking at the composition of canola meal and working back from about 40 % oil removal.

As an aside, here's the NREL algae biodiesel study link if anyone was looking for it.
07 October 2005
Integrated Biofuel Co-op
A big part of my shtick is that through a combination of conservation gains and offsetting load to electricity that it would be practical to produce and consume biofuels as our portable fuel of choice. Still I have to say to myself, "do the math stupid." Too many ideas attractive paper concepts don't stand up to rigorous analysis. As such I would like to do a systems analysis for an integrated biofuel farm setup. It would be run as a co-operative by many farmers who grow not just biodiesel but food and other cash crops.
An abstract diagram of the concept is below (click to enlarge):
Integrated Farm Biofuel Layout
For the moment I will use rapeseed (aka Canola) as my oil source, wheat as food, and clover as a nitrogen fixing rotation. Rapeseed appears to be the best crop for biodiesel production in Northern climates (ignoring algae schemes for now). Its yields are significantly below that of tropical palm kernel oil although that does not necessarily mean anything on an energy return basis.
By integrating the production locally I hope to realize higher well-to-tank efficiency for the farm machinery. A local biodiesel plant will reduce the cost of distribution for the farm tractors. Similarly the trucks that transship the farm producst to to a pipeline or train depot could fuel up literally at the well. The transesterification of biodiesel will require either ethanol (derived from seedcake) or methanol (derived from the biogas).
The seedcake and other farm waste goes into a set of solar ponds (not the salt gradient variety). Filled with methane farting bateria they mangent away on pretty much any organic material thrown their way. The biomass is slowly converted to methane gas which is then captured, compressed to a high pressure and stored on-site in tanks. The biogas can fill a number of local uses. It can be burned to provide energy inputs for biodiesel production. It can be used locally for home heating, irrigation pumps, etc. Or it can be shipped off in compressed natural gas tanker trucks. By being a net producer of natural gas the farm co-op should be insulated from variations in the price of fertilizer production. Ammonia and potash production are particularly dependant on natural gas.
Electricity is produced locally as well. Wind turbines are sited when viable, and linked to a local substation that does voltage regulation. A electricity storage system may be located on site to make the electricity production more reliable. A solid oxide fuel cell or steam turbine operates as a combined heat and power plant for the biodiesel production facility.
Writing the entire analysis in one sitting is totally infeasible. I have too much material to explore and learn. I would like to take a blog-style approach to the problem and break it up into its constituents and post them in turn.
04 October 2005
TheWatt Gets a Standing Eight-Count
We hope to have normal news service restored as soon as possible.
03 October 2005
Uber Atomic Battery
While Plutonium-238 can be bred in a nuclear reactor fairly easily, I did start to wonder if there's a better radioisotope out there.
As you can see from the nice diagram I stole from Hyperphysics the natural Thorium-232 chain does contain a nice radioisotope decay chain starting at Radium-228. The overall half-life of the chain is only about 8.6 years and it drops 20 amu over that time.
So how much power does Ra-228 contain? Well I get to go through a radioisotope table and add up the decay energies. It works out to 38.575 MeV along the most popular decay path. If we convert that to moles it works out to an impressive 3.72 TJ/mol, or 16.3 TJ/kg! This is more energy than fissioning Uranium can produce, but less than light element fusion.
The power density is quite impressive too. While the radioisotope starts off slowly with the beta decay of Ra-228 to Ac-228, it quickly picks up speed after a couple years. This is a curious advantage for a space probe: you don't want the RTG to be producing power on the ground because radiating the heat is difficult.
If you approximate the decay as chain as Ra-228 → Th-228 (half-life 6.7 years) and then Th-228 → Pb-208 (half-life 1.9 years) then it's fairly straightforward to find the power output. The power output is in excess of 20 kW/kg from about 1.5 years to 10 years. This is a minor 3300 % improvement over the Plutonium radiothermal generators.
Since the nuclear reactions here are alpha and beta decay, only charged particles are emitted. These are relatively easy to capture in magnetic fields and produce electricity from directly, as opposed to the neutron production in fission reactors. You would of course want to capture the energy emitted with a very high efficiency because radiating it is difficult. Also, the half-life is quite short and the decay ends as relatively harmless lead, so there's not much of a waste problem.
There are some obvious drawbacks. For one, the power output is so massive that a block of Ra-228 wouldn't exist as a solid -- it would produce enough energy to vapourize itself. Thin films could probably exist as solids. It also has Radon-220 in its decay chain -- a very unnice gas. Then there's also the point that it's the ultimate material for a radiological weapon. But these are only minor pettifogging details next to the production question.
While Ra-228 occurs naturally from natural Thorium-232, notice the 14 billion year half-life of Thorium. Given the short half-life of the decay chain, the preponderance of Ra-228 in natural Thorium will be incredibly low. Unless someone has a way of inducing alpha decay I don't know about, this leaves one alternative: transmutation.
Transmutation generally involves smacking protons into a material to make a heavier one. Looking down the periodic table there isn't a suitable atom for Radium transmutation until we get to Bismuth-209. The other elements (Po, At, Rn, Fr) don't occur in any significant natural abundance. A suitable light atom counterpart would be Fluorine-19. Here we have one advantage at least: Bismuth-209 and Fluorine-19 are both the only naturally occurring isotopes of those two elements. As a plus, both are cheap. On the negative side, the result would be Rutherforium-228 which isn't a known isotope and it would quickly fly apart. I.e. we have too many protons, not enough neutrons. Boron-20 simply isn't realistic. A better idea might be to smash deuterium nucleai into Ra-226 (half-life 1600 years), which would produce Ac-228 (the third element of the Thorium decay chain). This would certainly ramp up the power output at the expense of duration.
So the objective is to accelerate a positively charged ion up to some relativistic velocity and smash it into a heavy atom target to make Ra-228. There's two basic particle accelerators: the cyclotron and the synchrotron. The biggest cyclotrons in the world, like TRIUMF, can accelerate protons up to 500 MeV/amu with very high beam densities.
The synchrotron can do better. The Brookhaven Relativistic Heavy Ion Collider (RHIC) runs 100 GeV/amu and proton units at Fermilab and CERN are way bigger. The synchrotron has no real fundamental upper limit other that cost.
Of course, now if we start thinking the following question asks itself: if the ion acceleration energy is less than the 38.6 MeV we get from the decay chain, can we use this Earth-side to produce power? The critical observation is that we are spending kinetic energy to produce nuclear potential energy. The process is simply moving mass from two stable equilibria to an unstable equilibrium. The energy return rate could be enormously high if nature is kind (as if).
None of the processes involved has an inherent low efficiency. Particle accelerators use electromagnetic fields which transfer energy very efficiency. Radioisotope yields can be in excess of 90 %. The decay products are all charged particles, which can be efficiently captured or at least the system can produce high temperatures to drive a heat engine. Also realize that the kinetic energy of the ion is not lost when transmutation occurs. Instead it's converted to heat. That heat can be recovered and used to produce electricity, in effect reducing the power input for the accelerator. Check out this proposal for just that.
Unfortunately I have no idea how to estimate the energy needed for atomic fusion. It's probably significantly higher than 38.6 MeV or someone else would have already suggested the idea, right? As a potential power source for space applications it remains intriguing even if the energy balance is negative. There's also the possiblity that there's superior decay chains that I haven't noticed (Th-229 is good), especially those with higher Z than 240.
Zoom zoom...
02 October 2005
Coal is a Hydrocarbon (Sort Of)
ΔH(C) = -32.7 MJ/kg
ΔH(Coal) = -27.5 MJ/kg, % C = 65% by mass
therefore only 21.255 MJ/kg of energy from coal is derived from burning carbon. The remainder must come from either the combustion of Sulfur or Hydrogen. Sulfur is a fairly trivial component, being 1-2 % by mass and it only provides ΔH = -4.63 MJ/kg.
This means that roughly 27.5 MJ/kg - 21.25 MJ/kg = 6.2 MJ/kg of the heating energy is derived from Hydrogen. The only other potential energy source is Carbon double bonds but I have no way of estimating the frequency of those. Since the H-C bond and H-H bond are quite close in terms of energy I'll just assume that all of this energy is provided by the production of water at 141.9 MJ/kg. That gives a Hydrogen yield of 0.0437 kg(H2/kg(coal). This is surprisingly high -- it suggests my reference coal is almost the equivalent of Benzene. There's only 1.35 C atoms for every H atom. I am mildly suspicious of this result. It is in the right order of magnitude from this table for Australian coal.
This changes the chemical yield of coal gasification slightly. If %C = 0.65 and %H2 = 0.044 then each kilogram of coal yields 0.109 kg(H2) + 0.044 kg(H2) = 0.15276 kg (H2). This represents a 40 % increased chemical yield over my original coal gasification figures! The coal → Hydrogen yield is now 6.54 kg/kg.
Including heating input (7.2 kg/kg), the total coal input drops to 13.75 kg/kg. The fuel cost of Hydrogen derived from gasification drops from $0.72/kg to %0.61/kg. The CO2 production drops to 33 kg(CO2)/kg(H2).
We can run the comparisons to electrolysis at the two price levels once again:
Electricity Price | $40/MWh | $74/MWh |
Gasification Price delta | $1.49/kg | $3.28/kg |
Gasification CO2 Price (33 kg CO2:1 kg H2) | $45/ton | $100/ton |
The average improvement is about 25% over my previous calculations in favour of gasification. Further improvements to the thermal efficiency of the process will only make minor improvements in the cost of coal derived Hydrogen, but it will further depress the CO2 production and make it more difficult for electrolysis to compete.
29 September 2005
Oye'd! to Carbon
Coal Gasification
The chemical reaction for coal gasification isH2O + C → CO + H2, ΔH = +131 kJ
CO + H2O → CO2 + H2, ΔH = -41 kJ
So overall we turn 1 mole of Carbon into 2 moles of diatomic Hydrogen and 1 mole of Carbon Dioxide for an ideal energy input of 90 kJ.
Thus the ideal energy input is 47 kJ/mol of H2 = 22.32 MJ/kg of H2. The ratio of Hydrogen to Carbon by mass is 1 kg:5.96 kg.
Burning Carbon
Carbon is burned to Carbon Monoxide and then Carbon Dioxide:2C + O2 → 2CO, ΔH = - 221 kJ
2CO + O2 → 2CO2, ΔH = -566 kJ
Therefore pure carbon yields 393.5 kJ/mol = 32.76 MJ/kg
In contrast, coal is not nearly so good a fuel because it contains water and other contaminants. I picked Illinois bituminous coal at 11,800 Btu/lbs. This coal is equivalent to 27.5 MJ/kg. This is basically why coal is not considered a transport fuel. Note that this is basically the highest quality coal that we burn for fuel. The best stuff is saved for steel manufacture. The carbon content would be about 65 %. Oxygen and Nitrogen constituents take up many bond positions and lower the overall recoverable energy. Hydrogen adds some energy with much of that bound as water, and Sulfur a very tiny amount.
More info on coal available here:
http://www.eia.doe.gov/cneaf/coal/quarterly/co2_article/co2.html
Note this article states that Illinois bitluminous coal produces 203.5 lbs. of CO2/MBtu. With 11,800 Btu/lbs. the mass ratio coal:CO2 is 1:2.4013, which you can use to confirm the carbon content of 65 %.
Gasification Inputs
As mentioned before, the ideal input is 22.32 MJ of heat and 5.96 kg of Carbon to produce a single kilogram of Hydrogen gas.1. Heating input: 22.32 MJ of heat at 55 % cycle efficiency is 40.58 MJ. Given energy content of 5.63 MJ/kg, a total of 7.2 kg of coal must be burned for fuel. Now obviously the efficiency of this stage can be challenged.
2. Chemical input: 5.95 kg of carbon is needed, and the coal has 65 % Carbon content, for a total input of 9.154 kg. This stage should be around 99 % efficient.
TOTAL COAL INPUT = 7.2 kg + 9.15 kg = 16.35 kg of coal / kg of H2
Cost Input
Coal is priced at $40/short ton = $0.02/lbs. = $0.0442/kg.So our 16 kg of coal costs $0.723.
Obviously I made some sort of bad error in my last post. I will probably have to stop trying to run through multiple calculations using just my calculator since I'm generating too many lazy errors. :-) Of course, this took about five times as long to write.
CO2 Pollution Credits
With 16.35 kg of coal consumed and 65 % Carbon content the total carbon combusted is 10.63 kg. This works out to 38.94 kg of CO2. Recall, again, this is for one kilogram of H2. We can check this with the EIA derived ratio of 1 kg coal:2.4 kg CO2 → 39.25 kg of CO2 .I'll examine two potential electolysis costs. $40/MWh is ultra-cheap electricity derived from a high quality wind resource. $74/MWh was the average cost of electricity in the states for 2003. At $40/MWh electrolysis H2 is $2.10/kg; at $74/MWh it's $3.885/kg. The difference between gasified coal and electrolysis is $1.38/kg and $3.162/kg respectively. Obviously the cost of electricity is a very sensitive parameter!
The cost to buy CO2 credits to make up the difference is now $35.4/ton for the $40/MWh case and $81.2/ton for the $74/MWh case.
I can actually make up a formula for this to see how the cost of electricity varies the CO2 price point:
Cost CO2 credit = [1.346/tonMwh] PElec - $18.5/ton
where PElec is the price of electricity in $/MWh.
--
I'll take a look at steam reforming again later. I probably made the same error there. The electrolysis numbers are still correct.
27 September 2005
Hydrogen: What source?
I made the following assumptions:
- Electricity at $40/MWh from wind.
- Natural gas at $15/GJ.
- Illinois coal at 23.6 Mbtu/short ton, cost of $40.00 per short ton.
H2 Source | Ideal Energy Input (MJ/kg) | Expected Efficiency (%) | Real Energy Input (MJ/kg) | Unit Cost ($/GJ) | H2 Cost ($/kg) |
Electrolysis | 142 | 75 | 189 | 11.1 | $2.10 |
Methane | 20.5 | 55 | 37.3 | 15.0 | $0.56 |
Coal | 22.3 | 55 | 40.6 | 1.6 | $0.065 |
As we can see, the cost of coal-derived Hydrogen is vastly lower than that of methane or electrolysis derived Hydrogen. The question I want to know is, in some carbon-trading scheme like Kyoto, what rate does CO2 have to trade at for electrolysis to become viable?
I figured out the amount of CO2 produced from the combination of actual material input and the heating requirement. For example, methane, with a heating value of 55.7 MJ/kg has a heating input of 0.67 kg of methane per kg of Hydrogen (which is about 1.8 kg of CO2), and 22 kg of CO2 are produced from the actual chemical reaction to split the methane.
H2 Source | CO2 Produced (kg CO2 / kg H2) | Marginal CO2 Cost (relative to CH4) ($/ton) | Marginal CO2 Cost (relative to coal) ($/ton) |
Electrolysis | 0 | 62.1 | 42.0 |
Methane | 24.8 | 0 | 20.9 |
Coal | 48.5 | -20.8 | 0 |
As you can see, Methane is the winner even with its assumed high price. Carbon dioxide needs to be trading at $62/ton in order for electrolysis produced Hydrogen to be competitive. Obviously I'm being rather kind to Hydrogen by giving it cheap wind power and pushing the cost of natural gas well above the world average (if not so much above the North American average).
Note that the implications for sequesterization are clear. If CO2 can be sequestered for less than $60/ton, methane should remain the preferred feedstock for Hydrogen. If it can be sequestered for less than $20/ton, coal is the winner.
25 September 2005
New Digs
Works with Mozilla, Firefox, and Opera...
Update: Microsoft IE works fine at 1280 x 1024 resolution but not any others... Ergh.
A Rational Case for Biofuels
I think a picture is worth a thousand words, when it comes to the asinine arguments of the biofuel lobby:
Biofuels like biodiesel and ethanol represent a carbon neutral energy commodity. The plants they are produced from consume all the Carbon Dioxide liberated in burning them. Because they are hydrocarbons, they are fungible. They are energy dense, can be stored for long periods of time and are easily portable.
The greatest drawback of biofuels is that they cannot replace more than a small fraction of our current oil consumption. There simply isn't enough arable land available in the world to grow the crops that would be needed to fuel our oil habit. Realistically we might be able to replace 5 % of our current oil consumption with biofuels. Therein lies the inane nature of the biodiesel Hummer -- it may be renewable, but it is not sustainable.
My thought is, however, that we should not dismiss biofuels simply because their lobby groups are stupid. Instead, I think they have an important part to play as one component in a multi-faceted approach to the replacement of oil. The key is to exploit the portability of biofuels. In my viewpoint, biofuels and hydrogen are direct competitors as fungible fossil fuel replacements. Neither biofuels or hydrogen reduce our energy consumption.
In sharp contrast, an integrated approach to reduce the amount of energy we consume for personal transportation can increase the role of biofuels. To make biofuels significant we need to do the following:
- Conservation
- Superior well-to-wheel efficiency
- Offset load to electricity
Conservation incorporates a whole variety of factors to reduce consumption. Public transportation is one. Reduced speed limits with photo-radar enforcement is another. Reducing highway speed limits to 90 km/h could increase fuel economy by 15 %. Note that conservation is technology independent.
The biggest gain in conservation to be made is by switching to smaller cars. The current spat of SUVs are oversized beyond our needs. Mandating a switch to smaller vehicles, through something like a feebate, could drastically increase fuel economy. A fleet-wise mileage gain of 25 % from a progressive feebate is quite possible, and the impacts of rising gasoline prices will probably further assist it. Realistically, until GM goes bankrupt, we probably won't see a serious conservation effort in North America. As a huge employer General Motors welds great influence with politicians which allows them to continue their short-sited strategy of pushing giant vehicles. Toyota will pass GM as the world's largest car manufacturer soon enough.
I will assign conservation a 40 % decline in our per capita oil consumption.
The second step is to pick vehicle and energy sources technologies that produce a high well-to-wheel efficiency. The well-to-wheel efficiency of a spark ignition gasoline car is only about 13 %. Gains can be made by incorporating hybrid technologies like regenerative braking, auto-shutdown when stopped at red lights, storing energy from downhill runs for uphill climbs, etc. Switching to compression ignition (Diesel) combustion engines can also increase efficiency by 50 % over spark ignition. Studies have shown that a diesel-hybrid can have 3x the well-to-wheel efficiency of spark ignition engines.
As an aside, fuel cell car powered by electrolysis-produced hydrogen would have a similar well-to-wheel efficiency as gasoline cars. I.e. the hydrogen economy will not reduce our energy consumption.
The third step is to switch transportation load from portable energy sources like gasoline or biodiesel to electricity. This clearly lies in the gradual introduction of the plug-in hybrid (PHEV) with ever increasing all-electric range. A plug-in hybrid with a range of 30 km could easily replace 75 % of hydrocarbon consumption simply because most people don't drive that far in a day.
Electricity can more easily be produced from carbon neutral energy, whether it be renewables, nuclear, or sequestered clean coal. Moreover, the round-trip efficiency of electricity can be extremely high. If we consider power from wind, the electricity may lose 10 % of its energy to transmission losses; Li-ion batteries have a round trip efficiency of 90 %; electric motors can be 95 % efficient. The well-to-wheel efficiency in this case is a staggering 77 %. The plug-in hybrid also can act as grid voltage regulation, mitigating the intermittent aspects of renewable power sources like wind.
So what is the cumulative effect of these three ideas?
Conservation reduces our per capita normalized oil consumption from 1.0 to 0.6. We then replace 75 % of that load with electricity, reducing consumption to 0.15. Now calculate that the switch to the diesel-hybrid increases efficiency by a factor of three, so 0.15 / 3 = 0.05.
Guess what, through these three steps we have reduced our fossil fuel consumption to 5 % of its current value. Remember, how much off our current oil consumption we might be able to replace with biofuels? It's the same number. We can potentially replace all of our fossil fuel consumption for personal transport with biofuels. At the same time, energy consumption would be only 15 - 20 % of its current number, which has great implications for sustainability.
While this may be a simplistic analysis, it does show that biodiesel production could be on the same order of magnitude as consumption. Even if biodiesel and Fischer-Tropsch diesel from biogas can't meet all our oil consumption needs, fossil fuel consumption would be tiny compared to today. Carbon Dioxide emissions would be trivial in comparison, and peak oil would no longer be an issue.
Now, obviously this only addresses personal transportation and not freight transportation. Still, it shows that huge advances can be made in an incremental fashion to our current transportation industry.
21 September 2005
Thermochemical Hydrogen
I2 + SO2 + 2H2O → 2HI + H2SO4 (120 oC)
H2SO4 → SO2 + H2O + 0.5 O2 (850 oC)
2HI → I2 + H2 (300 - 450 oC)
As you can see the Sulfur and Iodine are recoverable, making this a recyclable process. The thermal efficiency of this cycle for nuclear power plants can be higher than the thermal efficiency of electricity production combined with electrolysis. For example, this detailed study found an ideal efficiency of 51 % (possibility higher with membranes) and an actual efficiency of 36 %. Such a reactor should still be capable of converting the remaining thermal power to electricity at around 30 % efficiency. Hence a thermochemical hydrogen reactor could potentially output 50 % hydrogen, 15 % electricity, and 35 % waste heat.
Hydrogen produced from nuclear power is a popular topic with Hydrogen economy advocates such as Dr. Ballard. However, there is a key drawback to using nuclear thermal energy for the Sulfur-Iodine process: most nuclear reactors simply don't operate at 850 oC. New reactors with novel cooling systems such as helium or sodium are necessary to produce the requisite temperatures.
While these numbers for the Sulfur-Iodine cycle may look impressive, I don't think hydrogen advocates are doing themselves any favours in pushing for new, untested nuclear technology. In reality, it represents another huge capital cost on the 'bridge' to a hydrogen economy.
The three main nails in the coffin of the hydrogen economy are:
- Well-to-wheel efficiency
- Storage
- Financial potential gap
Well-to-wheel efficiency is a major drawback of hydrogen. Principally, hydrogen does not reduce our energy consumption because its overall efficiency is similar to that of spark ignition gasoline vehicles. A diesel-hybrid, on the other hand, can increase efficiency by a factor of three. Hydrogen advocates often gloss over this problem, choosing to examine the high tank-to-wheel efficiency of fuel cells rather than the low well-to-tank efficiency of hydrogen production. I would submit, however, that as a portable, carbon-neutral energy source hydrogen is inferior to biofuels. After all, if we want hydrogen as a portable energy source, and we don't feel electricity storage is up to the task, why shouldn't we compare the energy return on energy investment (ERORI) of hydrogen to biofuels like corn-based ethanol or biodiesel?
Let's take David Pimetal of Cornell's study of ethanol production. This is widely regarded as one of the most hostile treatments of ethanol around. Even for corn, one of the worst sources of biofuel available, Pimetal finds an ERORI of 71 %. If every Joule of input into the production of corn-based ethanol was electricity, it would still have a superior ERORI to electrolysis-produced hydrogen. There's nowhere to go but up for biofuels, while hydrogen is stuck stationary due to the laws of thermodynamics.
Storage is a well known problem. Solid-state methods are generally no better than batteries; pressurized storage is potentially dangerous and consumes energy; cryogenic storage consumes a huge proportion of the heating value of hydrogen for the cooling process. Carbon-fuels -- whether from renewable sources or not -- have a much higher energy density while electricity can lay claim to far superior return rates (in excess of 90 % for Li-ion).
The financial potential gap is the chicken and egg paradox of infrastructure. No one can afford to build hydrogen fuel infrastructure until they have a market, and no consumer can afford to buy a hydrogen powered car without the supporting fuel infrastructure. This is in sharp contrast to incremental technologies like the hybrid. The hybrid has several paths where it can make incremental improvements in fuel economy and carbon economy while slowly evolving the existing infrastructure. It can improve the ancillary equipment, by transforming from mechanical belts and transmissions to electrical systems. It can enlarge the battery capacity, becoming a plug-in hybrid with increasing all-electric range with every generation. It can switch from spark-plug ignition to compression ignition (i.e. diesel). It can incorporate biofuels such as ethanol, biogas, or biodiesel into its tanks in increasing proportions. A fuel economy advances, it will become practical to advance from E5 to E20 to E40. Essentially, while the hydrogen economy faces a fiscal cliff, the hybrid only has to climb a set of stairs.