In the era of cheap energy the development of more efficient services (transportation, lighting, etc.) historically has not led to a reduction in consumption. While the standard in automobiles may have progressed from a blocky Ford V-8 POS to the stylish Honda Accord the corresponding increase in fuel economy has not reduced per capita gasoline consumption (caveat: for transportation, given the shift away from oil for electricity generation). Instead, people have taken advantage of the reduced marginal cost of driving to simply drive more. Alternatively, they might have taken their savings in energy in and sunk it into a February plane ticket to Mexico. Either way the annual kilometers traveled by the average person has increased, not decreased over the past century.
This is not so much a conscious decision people make to consume more energy but rather a move towards enriching ones lifestyle. Wealth is not the number of digits of fiat currency one has in their bank account and stock portfolio. That would be an overly simplistic viewpoint. The wealth of an individual is argely be determined by the services they have access to and can afford. By most measures I am wealthier than the Rockefellers of the late 19th century. I have access to faster and far safer transport, superior health care, and the marvel of modern internet communication, yet I am a graduate student and live below my country's version of the poverty line. All of my wealth is due to the efforts of scientists and engineers before me who have developed the technology and infrastructure that I now enjoy.
This issue becomes important when we try to evaluate the impact of improved efficiency on the production of greenhouse cases or oil consumption. Would a significant effort to increase the fuel efficiency of the personal vehicle fleet lead to a real reduction in oil consumption? Or would the reduction in demand drive a price drop in the cost of various forms of energy and in turn generate more wealth?
At what price point does efficiency begin to effect conservation positively?
I am not an economist (and economists have a terrible track record on predicting the future) but I suspect the answer lies somewhere between demand elasticity and fossil fuel depletion rates. I would suggest that the more inelastic the demand for oil the greater the long term reduction in consumption with increased prices. A high degree of inelasticity suggests that the system has a great deal of inertia and that much of the consumption is not so much voluntary but necessary to produce our wealth. In comparison shifts in elastic economy would be more temporary. Fossil fuel depletion is important because it dictates supply or will do so very shortly.
While improvements in efficiency certainly seem to present the lowest hanging fruit on the quest for sustainability they will likely only have a transient impact on energy consumption. Long term solutions to our energy situation will require a change in supply rather than demand.
Discussion regarding the art and science of creating holes of low entropy, shifting them around,
and then filling them back up to operate some widget.
28 November 2005
23 November 2005
Sam Passes On
Sam the Dog (see upper right-hand side of your screen) has passed on to a better place according to CNN. I can only hope that he's in a better place right now, cavorting with forty virgins in the fields of paradise.
sniff
In other news I have been catching up on my sleep since finishing my written thesis and all the bureaucracy associated with actually organizing my oral defense. Also Ben at theWatt.com has gone AWOL at the National Research Council labs so I have been trying to keep that site from becoming moribund. I promise to finish off some of the half-complete posts sitting in my box sometime soon. Of course, if my definition of soon is not the same as yours, too bad.
sniff
In other news I have been catching up on my sleep since finishing my written thesis and all the bureaucracy associated with actually organizing my oral defense. Also Ben at theWatt.com has gone AWOL at the National Research Council labs so I have been trying to keep that site from becoming moribund. I promise to finish off some of the half-complete posts sitting in my box sometime soon. Of course, if my definition of soon is not the same as yours, too bad.
13 November 2005
Embedded Energy Metric
Using thermodynamic laws it is usually fairly straightforward to analyze the efficiency of various systems of turning energy (more properly termed exergy) into work or heat. E.g. we can compare the ability of a heat pump next to electric resistance heaters to transform electricity into space heat. However it's often necessary to slide-slip away from the issue of the initial energy investment required to produce a baseboard heater compared to a heat pump because the data are proprietary or simply not gathered.
This energy investment in a product is known as the embedded energy. It is equivalent of capital investment in the money dimension. For example, in order to compare wind turbines to a combined-cycle thermal-electricity plant we should compare not only the CO2 offset by using wind rather than natural gas fuel to produce electricity but also the energy invested in the construction of the infrastructure as well. The steel and concrete in a wind turbine has an associated energy and CO2 production embedded into it during its manufacture. This embedded energy must be amortized over the lifespan of the turbine in order to properly determine the CO2 emissions that are offset.
The trick is how to determine the embedded energy in a product with adequate accuracy? To a certain extent this issue has stalled my investigation into the use of anerobic bioreactors to produce biogas from crop waste along with difficulties finding data on reaction rates as a function of temperature. Well, this being the blogosphere, an order of magnitude calculation on the back of an envelope should suffice. My thought is that if I could establish a metric for some common materials then I could simply estimate the embedded energy based on the mass of material in a product. The embedded energy problem is potentially simplified but it still remains challenging. Just try to find the mass of an air conditioner on a vendor's webpage, for example.
As a rough measure every material in the energy world can be subdivided into the following categories:
Rather than try to determine the energy embedded in a particular alloy I am hoping that StatsCan will have industry-wide figures for both production and energy consumption which will result in a more inclusive result. Hopefully I will be able to arrive at a reasonable set of metrics by the end of the week. Of course it would be desirable to actually check the results. Most likely there will be a need to introduce a fudge factor to account for the transformation of sheet steel into an automobile.
This energy investment in a product is known as the embedded energy. It is equivalent of capital investment in the money dimension. For example, in order to compare wind turbines to a combined-cycle thermal-electricity plant we should compare not only the CO2 offset by using wind rather than natural gas fuel to produce electricity but also the energy invested in the construction of the infrastructure as well. The steel and concrete in a wind turbine has an associated energy and CO2 production embedded into it during its manufacture. This embedded energy must be amortized over the lifespan of the turbine in order to properly determine the CO2 emissions that are offset.
The trick is how to determine the embedded energy in a product with adequate accuracy? To a certain extent this issue has stalled my investigation into the use of anerobic bioreactors to produce biogas from crop waste along with difficulties finding data on reaction rates as a function of temperature. Well, this being the blogosphere, an order of magnitude calculation on the back of an envelope should suffice. My thought is that if I could establish a metric for some common materials then I could simply estimate the embedded energy based on the mass of material in a product. The embedded energy problem is potentially simplified but it still remains challenging. Just try to find the mass of an air conditioner on a vendor's webpage, for example.
As a rough measure every material in the energy world can be subdivided into the following categories:
- Steel
- Aluminium
- Copper
- Plastic (polyethylene)
- Silicon
- Concrete
Rather than try to determine the energy embedded in a particular alloy I am hoping that StatsCan will have industry-wide figures for both production and energy consumption which will result in a more inclusive result. Hopefully I will be able to arrive at a reasonable set of metrics by the end of the week. Of course it would be desirable to actually check the results. Most likely there will be a need to introduce a fudge factor to account for the transformation of sheet steel into an automobile.
12 November 2005
Simple Solutions for Truck Freight Efficiency
I'm not one for "me-too" posts but it's been a couple of weeks now and I'm feeling the need to publish something. Green Car Congress carried a story about the development of a simple boat-tail attachment to a trailer that reduces the coefficient of drag of semi-tractor trailer vehicle by 0.12.
http://www.greencarcongress.com/2005/11/new_boat_tail_d.html
This just goes show how simple it could be to improve the efficiency of freight transport by truck. There's a number of other improvements that could be refited to existing truck fleets, like shrouding the tires and creating a flexible connector between the cab and trailer. Once it became clear that truck manufacturers weren't about to make improvements to the aerodynamics of their vehicles the government should have stepped in with legislation. Sometimes industries need a carrot to get them over a hill. In this case they need a kick in the ass to get them moving in the right direction.
http://www.greencarcongress.com/2005/11/new_boat_tail_d.html
This just goes show how simple it could be to improve the efficiency of freight transport by truck. There's a number of other improvements that could be refited to existing truck fleets, like shrouding the tires and creating a flexible connector between the cab and trailer. Once it became clear that truck manufacturers weren't about to make improvements to the aerodynamics of their vehicles the government should have stepped in with legislation. Sometimes industries need a carrot to get them over a hill. In this case they need a kick in the ass to get them moving in the right direction.
01 November 2005
Solar Thermal Cooling
Yes, I know it sounds like an oxymoron but just stick with me for a moment.
US peak electricity consumption occurs during the summer months when the demand for cooling is high and the efficiency of heat pumps drops with the higher ambient temperatures. Air conditioning constitutes approximately 15 % of annual US electricity consumption. However, the proportion of demand on peak days in hot states is in fact much higher.
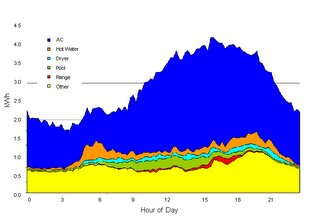
August day electrical consumption of a Florida home [Ref]
Supplying peak power is very expensive for utilities because they must build the infrastructure to cover peak demand which otherwise sits around underutilized. As a potential means of peak shaving we can examine the use of solar power to provide cooling. In this case utilities could be persuaded to offer businesses and home owners incentives to install such systems because it would improve their bottom line. The introduction of net metering with variable electricity prices could also provide an impetus. In the current environment of fixed rate electricity the consumer has little reason to purchase such a system.
There are two practical approaches:
A solar thermal system would use a collector to provide hot water for a single effect absorption chiller. Absorption chillers differ from common compression chillers in that cooling is driven by heat energy rather than mechanical energy. Aside from the plumping, several pieces of ancillary equipment are necessary including a controller, a pump, and a drain-back/expansion tank. Both systems are capable of providing hot water in addition to their cooling functions. For the PV system, a Heat Recovery Unit (HRU) would be used to pre-warm the water using waste heat from the heat pump.
Some further explanation of absorption chillers is warranted given that they are relatively uncommon. An absorption chiller transfers thermal energy from the heat source to the heat sink through an absorbent fluid and a refrigerant. The absorption chiller accomplishes its refrigerative effect by absorbing and then releasing water vapor into and out of a lithium bromide or ammonia/water solution. The process begins as heat is applied at the generator and water vapor is driven off to a condenser. The cooled water vapor then passes through an expansion valve where the pressure reduces. The low-pressure water vapor then enters an evaporator, where ambient heat is added from a load and the actual cooling takes place. The heated, low-pressure vapor returns to the absorber, where it recombines with the lithium bromide and becomes a low-pressure liquid. This low-pressure solution is pumped to a higher pressure and into the generator to repeat the process.
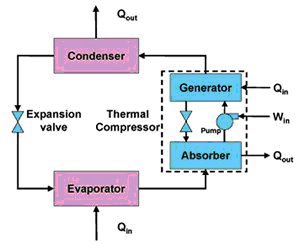
Schematic of Absorption Chiller Single-effect absorption chillers are not available for residential applications. Typically they are large units designed to operate off industrial waste heat. Small propane powered chillers are typically double-effect units that require a higher operating temperature than solar thermal collectors are capable of providing.
The performance of air conditioning systems is expressed by their coefficient of performance (COP). COP determines how many units of cooling/heating you get for every unit of energy you put in. Because units move heat the COP can be in excess of one. A typical value for a air conditioning heat pump is about 3.5 compared to only 0.7 for a absorption chiller.
The COP of an absorption chiller is heavily dependant on the applied temperature. If the solar thermal system is only capable of producing tepid water it will not function effectively. For my analysis I will assume that the absorption chiller requires an input temperature of 85 oC compared to the typically 55 - 60 oC required for residential hot water. The obvious conclusion from this is that the cheap unglazed flat-plate solar thermal systems used to warm pool water aren't going to provide the temperatures we need. Instead, we have to move up to evacuated tube collectors.
Evacuated tube solar thermal collectors are sold by companies such as www.apricus-solar.com. They incorporate a number of improvements to increase their efficiency and maximum operating temperature:
PV/Heat Pump: 0.125 * 3.5 = 0.44
Evacuated Solar-thermal/Absorption Chiller: 0.65 * 0.7 = 0.45
Amusingly they come out to being almost exactly the same. Assumably the inverter losses and parasitic heat losses are small and roughly equivalent. Presumably if you did an entropy analysis this would fall out quite naturally. Since the performance of each system is equivalent it is going to come down to cost.
If I size a 5000 W cooling system (for peak production at 1000 W/m2 insolation) for a home this is what I get:
This is ignoring mounts, plumbing and wiring for both the solar thermal collector and PV array. As we can see it is the cost of the evacuated solar collector pushing it above the PV solution. The expensive nature of evacuated solar thermal tubes is probably due to both their complexity and their low production volumes. Since they don't appear to be superior in this role now it seems unlikely that they would ever be able to catch up.
PV / Heat Pump System
Photovoltaic Panel
Name: Sharp ND-167U1
Peak Power: 167 W at 1000 W/m2 insolation
Module Efficiency 12.6 %
Area: 1.33 m
List Price: US$ 688.97
Heat Pump Chiller
Type: LG model LWK0710WGL
Cooling Power: 2051 W (7000 btu)
Coefficient of Performance: 3.43
Electrical Consumption: 600 W
List Price: US$ 330
Inverter
Type: Grid inter-tie
Name: Solectria PVI 1800W
Continuous Power Input Rating: 1980 W
Recommended Max PV Array Power: 2200 W
Continuous Power Output Rating: 1800 W
List Price: US$ 1731.25
Solar Thermal / Absorption Chiller System
Solar Collector
Name: Apricus 30 Tube Collector
Gathering Area: 2.4 m2
Estimated Peak Power Generation: 1536 W
Absorption Chiller
Name: Yakaza WFC-10
Cooling Power: 10 tons
Coefficient of Performance: 0.7
List Price: unknown (generally 2x that of heat pump)
Controller
Name: Apricus Sentinel-Pro controller
List Price: US $395
Pump
Name: Grundfos Stainless UP15-18SU
Power: 1/25 hp
List Price: $184
Drain-back/Expansion Tank
Name: Alternate Energy Technologies DB-10
Capacity: 10 gallons
List Price: $322
US peak electricity consumption occurs during the summer months when the demand for cooling is high and the efficiency of heat pumps drops with the higher ambient temperatures. Air conditioning constitutes approximately 15 % of annual US electricity consumption. However, the proportion of demand on peak days in hot states is in fact much higher.
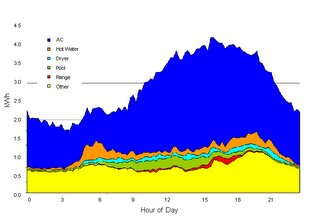
Supplying peak power is very expensive for utilities because they must build the infrastructure to cover peak demand which otherwise sits around underutilized. As a potential means of peak shaving we can examine the use of solar power to provide cooling. In this case utilities could be persuaded to offer businesses and home owners incentives to install such systems because it would improve their bottom line. The introduction of net metering with variable electricity prices could also provide an impetus. In the current environment of fixed rate electricity the consumer has little reason to purchase such a system.
There are two practical approaches:
- Pair a photovoltaic array with a standard air conditioning unit.
- Pair a solar thermal collector with an aborption chiller.
A solar thermal system would use a collector to provide hot water for a single effect absorption chiller. Absorption chillers differ from common compression chillers in that cooling is driven by heat energy rather than mechanical energy. Aside from the plumping, several pieces of ancillary equipment are necessary including a controller, a pump, and a drain-back/expansion tank. Both systems are capable of providing hot water in addition to their cooling functions. For the PV system, a Heat Recovery Unit (HRU) would be used to pre-warm the water using waste heat from the heat pump.
Some further explanation of absorption chillers is warranted given that they are relatively uncommon. An absorption chiller transfers thermal energy from the heat source to the heat sink through an absorbent fluid and a refrigerant. The absorption chiller accomplishes its refrigerative effect by absorbing and then releasing water vapor into and out of a lithium bromide or ammonia/water solution. The process begins as heat is applied at the generator and water vapor is driven off to a condenser. The cooled water vapor then passes through an expansion valve where the pressure reduces. The low-pressure water vapor then enters an evaporator, where ambient heat is added from a load and the actual cooling takes place. The heated, low-pressure vapor returns to the absorber, where it recombines with the lithium bromide and becomes a low-pressure liquid. This low-pressure solution is pumped to a higher pressure and into the generator to repeat the process.
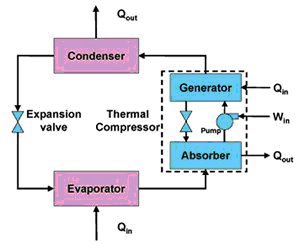
The performance of air conditioning systems is expressed by their coefficient of performance (COP). COP determines how many units of cooling/heating you get for every unit of energy you put in. Because units move heat the COP can be in excess of one. A typical value for a air conditioning heat pump is about 3.5 compared to only 0.7 for a absorption chiller.
The COP of an absorption chiller is heavily dependant on the applied temperature. If the solar thermal system is only capable of producing tepid water it will not function effectively. For my analysis I will assume that the absorption chiller requires an input temperature of 85 oC compared to the typically 55 - 60 oC required for residential hot water. The obvious conclusion from this is that the cheap unglazed flat-plate solar thermal systems used to warm pool water aren't going to provide the temperatures we need. Instead, we have to move up to evacuated tube collectors.
Evacuated tube solar thermal collectors are sold by companies such as www.apricus-solar.com. They incorporate a number of improvements to increase their efficiency and maximum operating temperature:
- The absorber is insulated from the air by a vacuum. This greatly decreases convective losses to the wind.
- The absorber is coating with a spectrally selective surface. Such a surface has a high absorption coefficient (α ~ 0.9 – 0.95) in the visible wavelengths (550 nm) but a low emissivity coefficient (ε ~ 0.02 – 0.08) in the far infra-red (10,000 nm). This reduces radiation losses to a minimum.
- Heat is transferred from the absorber to the working fluid by means of a heat pipe. A heat pipe is filled with a partial pressure of water, such that it boils at 30 °C. When the water evaporates, it convects up to a heat exchanger where it condenses, transferring its energy to the working fluid. The effective thermal conductance is roughly 10,000 times that of a solid copper bar. The heat pipe also has a diode function, in that if it is not hot enough to evaporate the water, no convection will occur.
PV/Heat Pump: 0.125 * 3.5 = 0.44
Evacuated Solar-thermal/Absorption Chiller: 0.65 * 0.7 = 0.45
Amusingly they come out to being almost exactly the same. Assumably the inverter losses and parasitic heat losses are small and roughly equivalent. Presumably if you did an entropy analysis this would fall out quite naturally. Since the performance of each system is equivalent it is going to come down to cost.
If I size a 5000 W cooling system (for peak production at 1000 W/m2 insolation) for a home this is what I get:
Photovoltaic | Solar Thermal | ||
Component | Normalized Cost (US$/Wcool) | Component | Normalized Cost (US$/Wcool) |
PV Array | 1.21 | Solar Collector | 1.99 |
Heat Pump | 0.16 | Absorption Chiller | 0.32 |
Inverter | 0.34 | Pump | 0.037 |
| Controller | 0.079 | |
Drain-Back Tank | 0.064 | ||
Total | 1.71 | Total | 2.49 |
This is ignoring mounts, plumbing and wiring for both the solar thermal collector and PV array. As we can see it is the cost of the evacuated solar collector pushing it above the PV solution. The expensive nature of evacuated solar thermal tubes is probably due to both their complexity and their low production volumes. Since they don't appear to be superior in this role now it seems unlikely that they would ever be able to catch up.
PV / Heat Pump System
Photovoltaic Panel
Name: Sharp ND-167U1
Peak Power: 167 W at 1000 W/m2 insolation
Module Efficiency 12.6 %
Area: 1.33 m
List Price: US$ 688.97
Heat Pump Chiller
Type: LG model LWK0710WGL
Cooling Power: 2051 W (7000 btu)
Coefficient of Performance: 3.43
Electrical Consumption: 600 W
List Price: US$ 330
Inverter
Type: Grid inter-tie
Name: Solectria PVI 1800W
Continuous Power Input Rating: 1980 W
Recommended Max PV Array Power: 2200 W
Continuous Power Output Rating: 1800 W
List Price: US$ 1731.25
Solar Thermal / Absorption Chiller System
Solar Collector
Name: Apricus 30 Tube Collector
Gathering Area: 2.4 m2
Estimated Peak Power Generation: 1536 W
Absorption Chiller
Name: Yakaza WFC-10
Cooling Power: 10 tons
Coefficient of Performance: 0.7
List Price: unknown (generally 2x that of heat pump)
Controller
Name: Apricus Sentinel-Pro controller
List Price: US $395
Pump
Name: Grundfos Stainless UP15-18SU
Power: 1/25 hp
List Price: $184
Drain-back/Expansion Tank
Name: Alternate Energy Technologies DB-10
Capacity: 10 gallons
List Price: $322
Subscribe to:
Posts (Atom)